Cancer is the second leading cause of death after cardiovascular diseases in almost all countries, with a trend of the incidence steadily increasing. Traditional approaches in cancer therapy (chemotherapy, surgery and radiotherapy) have been the workhorses for decades, but most of them are associated with side effects, resistance, or recurrence after initial treatment.
Nanomedicine offers new therapeutic approaches with high potential to be more effective and/or less invasive to patients. Our main goal is to develop a unique combination of internal radiation therapy and heating by using a single nanoconstruct composed of coated magnetic nanoparticles (MNPs) labeled with therapeutic radionuclides. As generators of both local magnetic hyperthermia (MH) and local radiotherapy (RT), they will boost cancer combat by synergism or a cumulative effect.
Research activities within this topic can be subdivided as follows:
(i) Search for MNP materials with high SAR values
The ability of MNPs to generate heat when exposed to an AC magnetic field is utilized for tumor destruction by its local heating in magnetic hyperthermia therapy. The ideal nanoparticle material should be biocompatible/nontoxic while possessing a high SAR (Specific Absorption Rate) value so to minimize the administered amount. The search for such material is still an ongoing effort worldwide. Our research deals mainly with iron oxides and ferrites, with the attempts to improve the SAR value by altering either morphological properties (size, shape, agglomeration) or composition by substituting iron for different cations (Fe3-xMxO4, M = Zn, Mn, Mg, Er, Y), Figs. 1 and 2. Different synthesis methods have been applied to control the size/crystallinity /size distribution of MNPs.
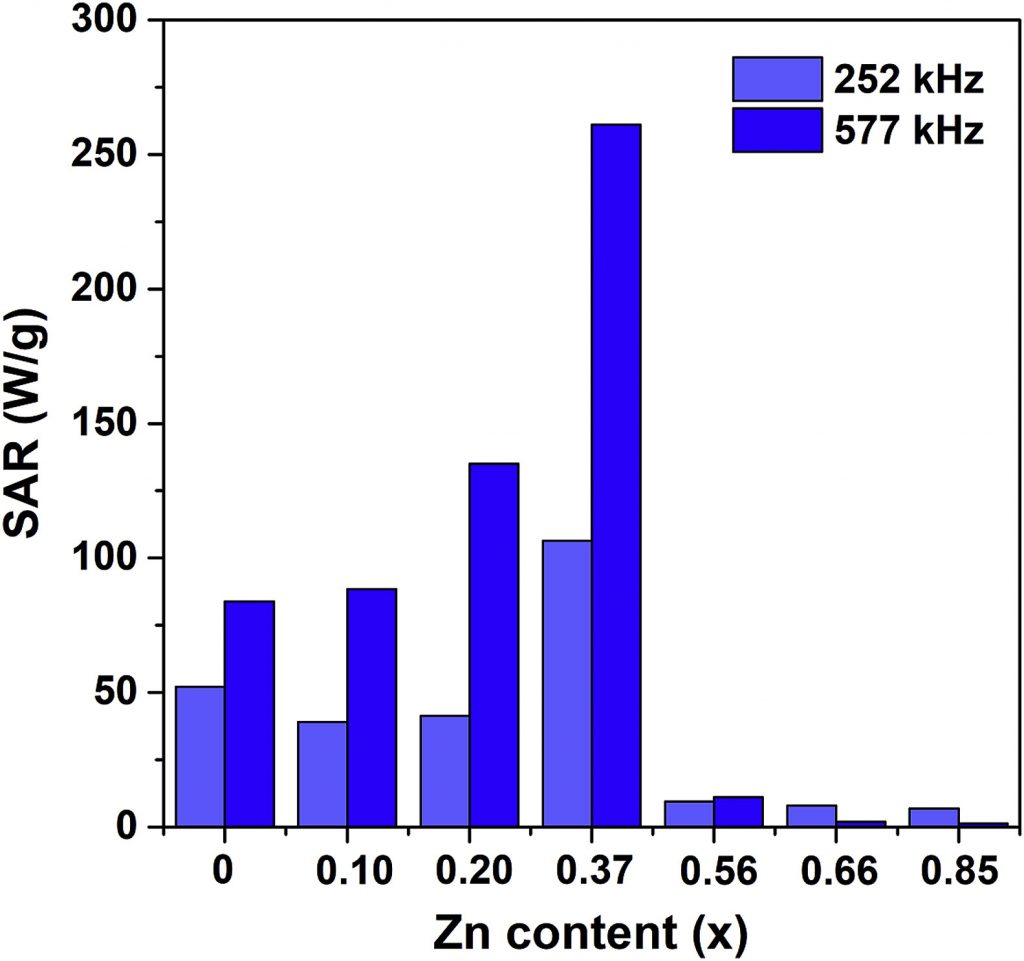
(M. Ognjanovic et al., J. Alloys Compd., 2019, 777, 454-462)
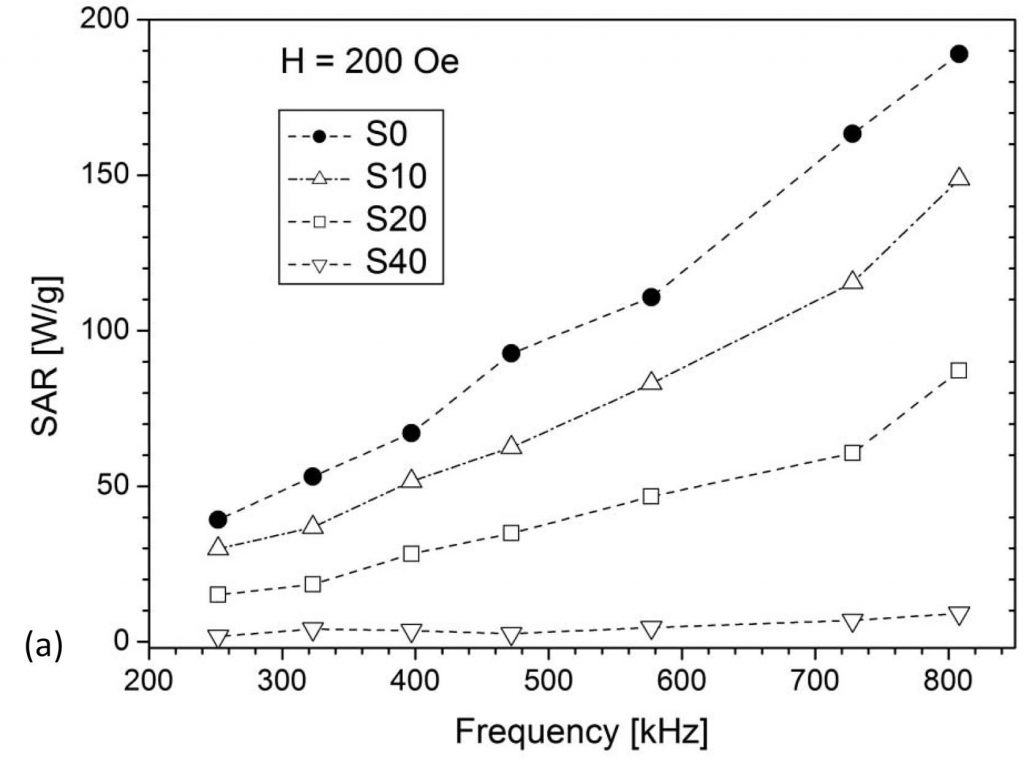
(V. Kusigerski et al., J. Magn. Magn. Mater., 2019, 475, 470-478)
(ii) Non-covalent interactions in crystal structures of molecules with potential application in medicine
Non-covalent interactions play a key role in the stabilization of the three-dimensional structure of biomolecules (proteins and nucleic acids) as well as in mutual recognition and interaction of these systems with the small biologically active molecules. Non-covalent interactions also have decisive role in the stabilization of the crystal structures of synthesized molecules and define their conformation in the solid-state. The aim of our study is to investigate the structural properties of small biologically active molecules and to analyze the structural features of molecular fragments important for the formation of non-covalent interactions in the crystal.
The study includes several aspects:
- Determination of crystal structure of small bioactive molecules, analysis of the molecular structure and interactions in the crystal state through the classical single-crystal X-ray diffraction experiments [1].
- Statistical analysis of the structural data deposited in the Cambridge Structural Database, with the aim to investigate preferable geometry of non-covalent interactions in the related classes of compounds [2].
- Determination of energy of non-covalent interactions in selected dimers and clusters of molecules by employing the software for quantum-chemical calculations [2,3].
Determination of the electronic structure of the small bioactive molecules through the high-resolution X-ray diffraction experiments in order to elucidate the structure-activity relationship at the subatomic level. The analysis includes: experimental determination of the total electron density of molecules through the Hansen-Coppens multipole model; topological analysis of the electron density and quantitative characterization of chemical bonds and interactions through the Bader’s quantum theory of atoms in molecules (QTAIM); determination of the electrostatic potential and identification of molecular regions relevant for chemical reactivity and processes of molecular recognition [3].
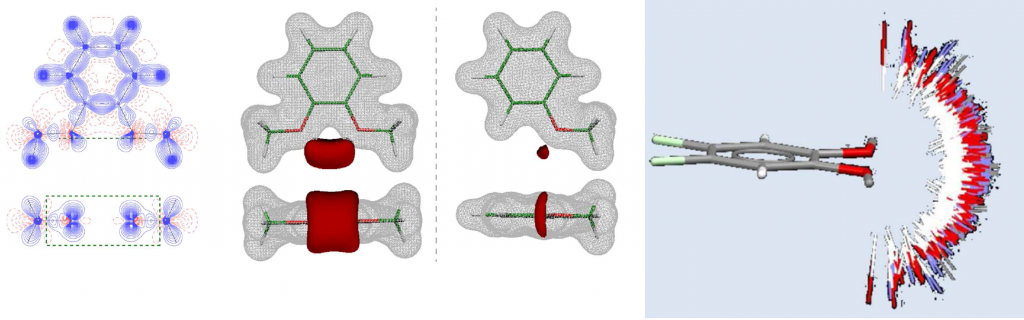
Selected references:
[1] M. Pešić, J. Bugarinović, A. Minić, S. B. Novaković, G. A. Bogdanović, A. Todosijević, D. Stevanović, I. Damljanović: Electrochemical characterization and estimation of DNA-binding capacity of a series of novel ferrocene derivatives. Bioelectrochemistry, 132, 2020, 107412.
[2] G. A. Bogdanović, B. Ostojić, S. B. Novaković: Short Intramolecular O···O contact in some o-dialkoxybenzene derivatives generates efficient hydrogen bonding acceptor area. Cryst. Growth Des., 18, 2018, 1303. [3] B. M. Francuski, S. B. Novaković, D. D. Francuski, G. A. Bogdanović: Charge density analysis of 2-pyridineformamide N(4)-methylthiosemicarbazone (Z′ = 4): Role of an enhanced N-H⋯S thioureido dimer. Cryst. Growth Des., 17, 2017, 2998.
(iii) Investigation of nanoparticles biodistribution
Of great importance in the evaluation of the usability of specific MNPs in theranostics is their biodistribution and pharmacokinetics. One of the important challenges regarding this research is to reliably quantify the biodistribution of exogenous iron oxide-based MNPs in the presence of the background signal originating from very abundant endogenous iron ions. One possible way to overcome difficulties in studying the biodistribution of iron oxides-based nanoparticles is to partially substitute Fe cations with 4f elements, which are not typically found in living organisms. Another possibility to study biodistribution patterns is to utilize a radiotracer technique, in which the distribution of radiolabeled MNPs after their intravenous administration is determined by measuring radioactivity in tissues and/or organs. In a work by B. Antic et al. (Materials Science and Engineering C 75,2017,157–164) comparison between ion coupled optical emission and mass spectrometry (ICP-MS) with radiotracer method has been done in studying the biodistribution of 90Y-labeled superparamagnetic citric acid (Fe,Er)3O4 coated nanoparticles on the animal model (Wistar rats), Fig. 4.
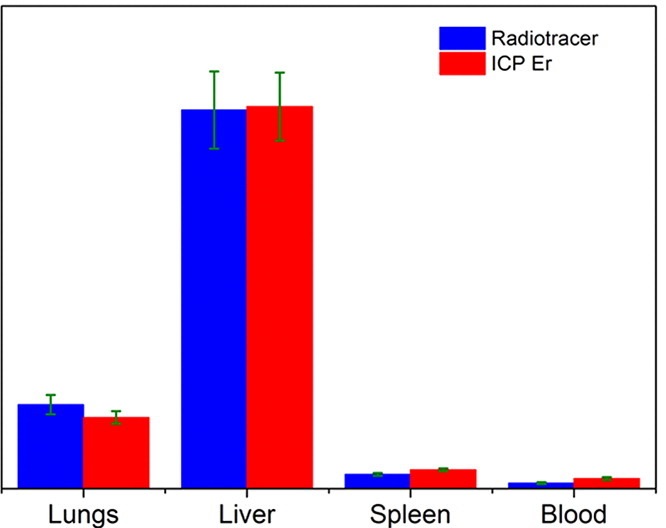
We have also applied a recently emerged technique for biodistribution studies which utilizes AC susceptometry for the determination of MNP’s concentration in tissues. It has been shown that a huge difference in dynamical magnetization response of endogenous and exogenous iron enables detection of superparamagnetic MNPs in tissues by measurements of imaginary part χ” of AC susceptibility. This method relies on relative measurements which means that one must first perform measurements on calibration samples which are ferrofluids with different concentrations of Fe. An example of MNPs biodistribution determination by utilizing AC measurements is shown in Fig. 5.
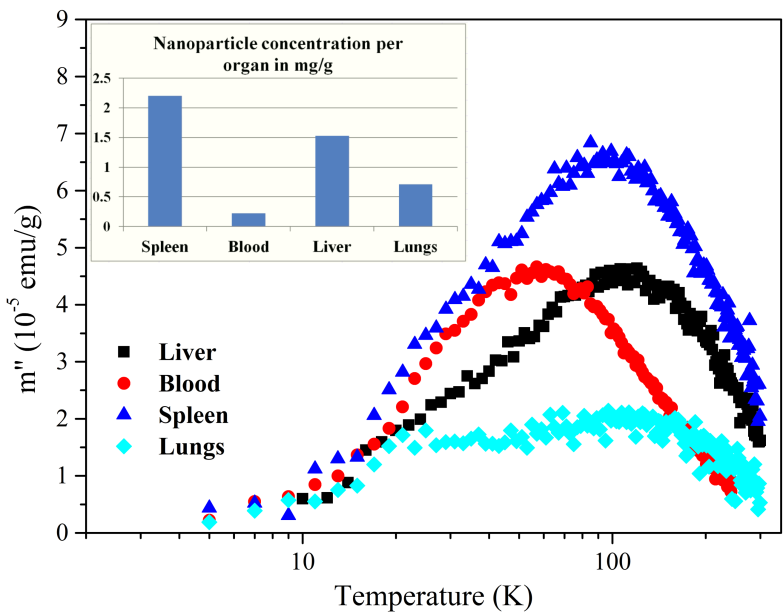
(M. Boskovic, PhD thesis Magnetic nanoparticles in medicine: measurement of specific losses and biodistribution, in progress).
(iv) Bimodal hyperthermia/radionuclide therapy
In the last decade a new approach, termed nano-brachytherapy, which combines advantages of brachytherapy and nanotechnology, is under investigation). It is based on the development of a radionuclide-loaded nanomaterial suitable for intratumoral application. The advantages of such an approach are low invasiveness, chance to treat inoperable tumors, safety, low side-effects, and low cost. In our first study, we have prepared a multifunctional 99mTc–bisphosphonate–coated magnetic nanoparticles (MNPs) based on Fe3O4 and coated with two hydrophilic bisphosphonate ligands, i.e., methylene diphosphonate (MDP) and 1–hydroxyethane-1,1- diphosphonate (HEDP). The presence of the bisphosphonates on the MNPs surface, enabled their biocompatibility, colloidal stability and successful binding of the radionuclide, Fig. 6.
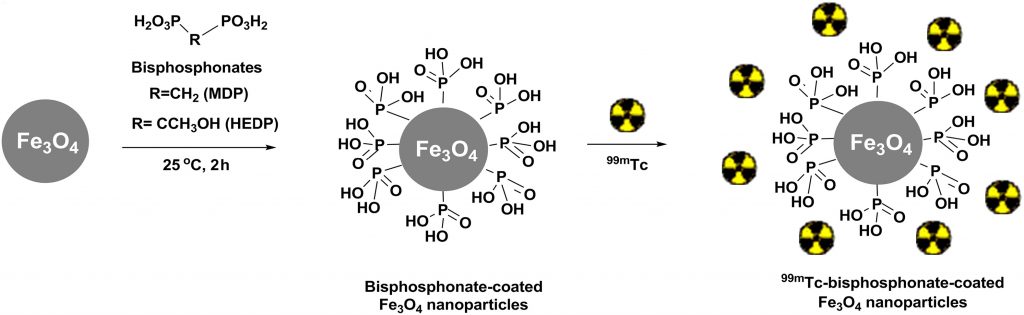
(M. Mirkovic et al., Mater. Sci. Eng. C, 102 (2019) 124–133).
The specific power absorption values for Fe3O4-MDP and Fe3O4-HEDP were 113 W/g and 141 W/g, respectively, indicated their heating ability under the applied magnetic field. Coated MNPs were radiolabeled with 99mTc using stannous chloride as the reducing agent in a reproducible high yield (over 95%) and were remained stable in saline and human serum for 24 h. Ex vivo biodistribution studies presented significant liver and spleen uptake in healthy Wistar rats after intravenous administration at all examined time points due to the colloidal nature of both 99mTc–MNPs. Results of scintigraphy studies are in accordance with ex vivo biodistribution studies (Fig. 7.), demonstrating high in vivo stability of radiolabeled MNPs. Overall, in vitro and in vivo stability as well as heating ability, indicate that biocompatible radiolabeled bisphosphonate MNPs exhibit promising potential as a theranostic nanoagent.
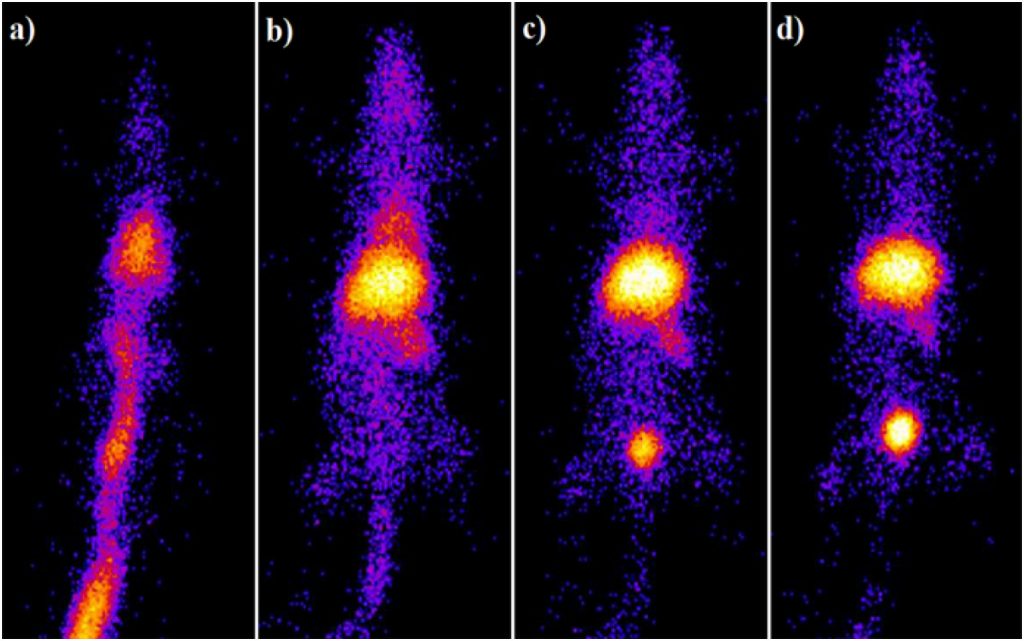
In the next study, we envisioned a complex nano-radiopharmaceutical, combining a radionuclide for radiotherapy, magnetic nanomaterial for generating hyperthermia, and an antibody to help to retain the material in the tumor, eventually maximizing the expected antitumor effect. APTES@SPIONs coupled to 131I-labeled CC49 mAb were successfully prepared, characterized and investigated as a potential multifunctional agent for combined radionuclide-hyperthermia tumor therapy. The APTES coating secures the stabilization by strong electrostatic repulsion and prevents the coated SPIONs from aggregation. The detected temperature of up to 46 °C achieved in vivo, confirms the preserved heating capability of 131I-CC49-APTES@SPIONs required for hyperthermia therapy. The choice of radionuclide 131I for radiolabeling was done based on its suitable energy, penetrability through tissue and half-life of 8 days. It well complements hyperthermia due to long-term retention of the injected material achieved by the introduction of CC49 mAb. After intratumoral injection into LS174T human colon adenocarcinoma xenografts on NOD-SCID mice, the retention study indicates prolonged tumor retention of nanoparticle’s complex even after 14 days. The results demonstrate the feasibility and effectiveness of magnetic hyperthermia, radiation and their combination in treating cancer in xenograft models. The combined therapy approach induced a significant tumor growth suppression in comparison to the control groups and it was better than radionuclide or hyperthermia treatment alone, Fig. 8. Besides, histopathology analyses demonstrate damage to treated tumors at the cellular level without causing a toxic effect.
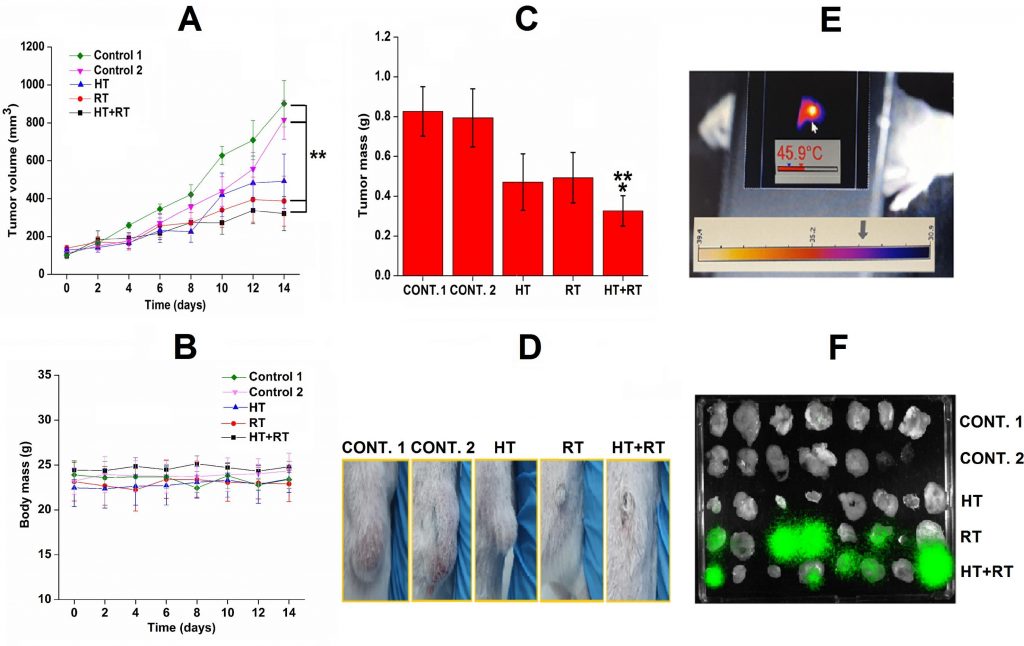
(A. Stankovic et al, Int. J. Pharm., 587 (2020) 11962).